By Tim Hornyak
The field of bio-engineering replacement body parts has come a long way from the 400-kilogram iron lungs of yore. Still, because artificial cells don’t fare well deep within large tissues, we’re not yet at the point of engineering big-ticket organs. For five McGill research teams, however, the collective thinking is that the path to “going big” starts with going small. Very, very small. Their tiny creations stand to make a huge difference in many people’s lives.
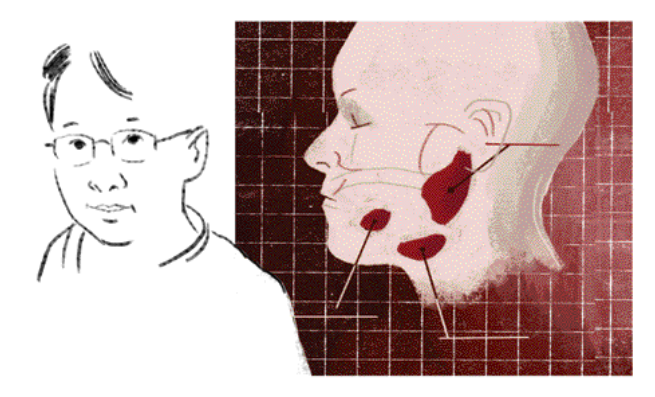
The next time you spit, spare a thought for those who can’t. Simon Tran of the Faculty of Dentistry has spent years thinking about how to help thousands of people suffering from severe dry mouth, loss of taste or mouth ulcers. There are several possible reasons behind these conditions (the most common are head and neck cancer, and their attendant radiation treatments, and the autoimmune disease Sjogren’s syndrome) and one common reality: There is no effective treatment. Tran is researching how to fix salivary glands with a two-pronged approach — by using bone marrow stem cells, and by bio-engineering new salivary glands. In the first approach, Tran has tested bone marrow cells for their ability to restore saliva flow in mouse models. He has also confirmed that human stem cells can repopulate salivary glands in leukemia patients.
The mechanism, however, remains unclear. When bones become fractured or diseased, a graft may be in order. Surgeons often use “autograft” bone from a patient’s own hips or ribs in the hope that the transplanted bone will reform and heal properly. But this procedure isn’t a panacea. The amount of bone that can be harvested is limited and numerous complications can arise; when bone from cadavers is used, it carries the risk of generating an immune response.
“The next step is to understand how the bone marrow stem cells provide this therapeutic effect of restoring saliva flow,” says Tran, who successfully cultured functional human salivary cells in 2005. Meanwhile, he and colleagues have built a prototype artificial salivary gland, and have managed to coat it with human salivary cells. Formed from a biodegradable substrate, the device is like a tube containing salivary cells, and would rest in a surgically created pouch in the mouth. They are now undergoing the tricky business of testing how well the seeded cells move water — a crucial step in saliva production.
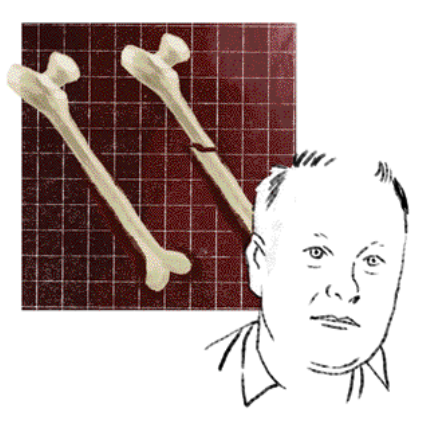
The Faculty of Dentistry’s Jake Barralet is developing materials to replace bone autograft. He’s concentrating on 3D graft printing using equipment similar to an inkjet printer. Materials such as calcium phosphate are deposited in layers, forming a 3D shape. The process includes the use of ions such as copper to stimulate tissue repair. “Biomaterials is like cooking—there is a finite number of ingredients and one becomes very skilled in squeezing new flavours and textures from them,” says Barralet. “We also have the skills and experience to take our discoveries through to practice and have an excellent technology conversion rate.”
When bones become fractured or diseased, a graft may be in order. Surgeons often use “autograft” bone from a patient’s own hips or ribs in the hope that the transplanted bone will reform and heal properly. But this procedure isn’t a panacea. The amount of bone that can be harvested is limited and numerous complications can arise; when bone from cadavers is used, it carries the risk of generating an immune response. The Faculty of Dentistry’s Jake Barralet is developing materials to replace bone autograft. He’s concentrating on 3D graft printing using equipment similar to an inkjet printer. Materials such as calcium phosphate are deposited in layers, forming a 3D shape. The process includes the use of ions such as copper to stimulate tissue repair. “Biomaterials is like cooking—there is a finite number of ingredients and one becomes very skilled in squeezing new flavours and textures from them,” says Barralet. “We also have the skills and experience to take our discoveries through to practice and have an excellent technology conversion rate.”
McGill has licensed the technology to biotech firm Bonegrafix, which aims to make solid bone blocks based on patient CT scans, to fill in holes in bones or to bind parts during healing. The commercial application may be an early step on the road to widespread clinical use. “One day in the near future, just as custom crowns and bridges are made for your dentist, so custom bone grafts will be available too.”
If we’re to custom-make replacement tissue, it makes sense to start with collagen, the dominant supportive protein found throughout the body. Collagen hydrogels are already widely used in tissue engineering, but researchers Showan Nazhat of the Department of Mining and Materials Engineering and Marc McKee and Mari Kaartinen of the Faculty of Dentistry are looking into how to quickly form dense nanofibrillar collagen matrices—and then strengthen them by biochemical cross-linking, seed them with a patient’s own cells and, ultimately, anchor them (by calcification) where they’re needed in the body. The technology could be used to replace ligaments, bone and cartilage, as well as to produce graft materials to treat bone and tooth defects (whether the result of trauma, cancer or cancer treatment).
“You can’t improve on biological evolution, but if you have a body part defect, you can make a bio-engineered replacement exactly to the right specs and not rely on another patient or harvesting replacements from other body sites,” says McKee. In vivo studies in animal models are still underway but, because collagen is a widely used and approved material for implantation into patients, the research could have human clinical uses in as little as five years. “By increasing the amount and quality of protein, we can produce a physiologically relevant matrix with increased mechanical competence,” says Nazhat. This durable end product, he hopes, will hold up to being handled, and sutured, by surgeons. “I’m not claiming we’re making perfect ligaments, for example, but what we’re making is something that will ultimately lead to a lifelike tissue.”
In 1957, while still an undergraduate, Thomas Chang invented the world’s first artificial blood cell —in his McGill dorm room, no less. His ingenious creation was a cell-like ultrathin polymer membrane that held hemoglobin. But the world wasn’t particularly interested in artificial blood cells at the time, so Chang largely shifted his concentration onto artificial cells that could be customized to contain other substances: enzymes, drugs and a host of other materials used in medicine and biotechnology. That research formed the basis for micro- and nano-systems (used in everything from regenerative medicine to gene therapy to drug delivery) that are being explored around the world. But, as HIV became a global health concern, Chang returned to his original blood work. And with good reason.
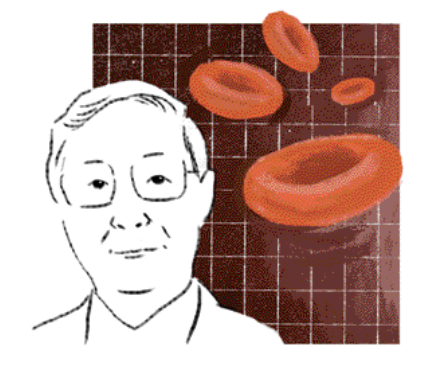
The HIV-tainted blood crisis of the late 1980s made Chang acutely aware of the need for a better artificial blood cell. In the understandable rush to create an alternative blood source, industry focused only on creating a substance that transported oxygen; the result was an imperfect product that led to serious side effects (such as constricted blood vessels) and even death. Chang, director of McGill’s Artificial Cells and Organs Research Centre and a recipient of the Order of Canada, is focusing on developing a blood substitute with all the functions of red blood cells— not just oxygen-carrying capabilities. By building on his original method of cross-linking a number of hemoglobin molecules together into soluble nanodimension polyhemoglobin, he’s creating cells that carry carbon dioxide as well as oxygen, and remove oxygen radicals, among other functions. These “PolyHb” cells do not have a blood group, which means they can be given to a patient immediately without knowing their blood type—and, in an emergency situation, can delay the need for donor blood by up to 12 hours. The cells can also be stored at room temperature for more than a year. Donor blood, in contrast, must be refrigerated—and, even then, only lasts for 42 days. South Africa has approved PolyHB for routine use in patients and Russia has more recently followed suit. North American approval is still pending.

When all goes well, an implant, be it a replacement hip or a tooth, readily integrates into its new host. But when things go awry, the implant causes discomfort or infection, and may need to be replaced. According to Marta Cerruti, success (or failure) hinges on what happens very early on. “Within a few minutes of an operation, proteins start to adhere to the surface of the implant,” explains Cerruti, an assistant professor in the Department of Mining and Materials Engineering and the newly appointed Canada Research Chair in Bio-synthetic Interfaces. “If the right proteins adhere, then all is well. But if the wrong proteins adhere to the implant, the body forms a fibrous capsule around the implant to isolate it and a new implant needs to be inserted.” Cerruti wants to find a better way for implants—whether for bone or soft tissues—to integrate within people’s bodies. The answer, she believes, lies in building functional, biodegradable scaffolds using a combination of organic materials and polymers. “The difficult part is directing the cells to do what we want them to do, whether that’s making skin or making bone,” she says. “The synthetic material should be able to communicate to the living, biological material how to react.” Each porous scaffold surface is covered with molecules particular to the task at hand; a bone implant, for example, would require molecules that encourage the formation of hydroxyapatite, the mineral component of bones. As the point of first contact between the host body and the implant, these molecules would act as biological signals and attract the desired type of proteins and cells. Then, when their work is sufficiently underway, the scaffolds simply dissolve away. (A skin implant scaffold might dissolve after a few weeks. For a longer process, like growing new bone, the scaffold would stay intact for months.) “We don’t want to just hope that the body will do what we want it to,” says Cerruti. “We’re directing it to do what we want it to by making materials that speak the body’s language.”